Articles
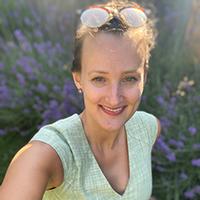
Ubiquity
Volume 2021, Number July (2021), Pages 1-12
How Does a COVID mRNA vaccine really work? an interview with Dr. Evelyn Tichy
Walter Tichy
DOI: 10.1145/3477388
The most potent weapon against COVID-19 is a vaccine based on messenger RNA (mRNA). The first of these vaccines authorized for use was developed by the German company BioNTech in cooperation with Pfizer, closely followed by the (U.S.-produced) Moderna vaccine. These vaccines send a piece of mRNA into cells of a host. The mRNA instructs the cells to produce masses of the same spike protein that also occurs on the shell of the real coronavirus. The immune system responds by learning to destroy anything showing that protein: if the real virus arrives, the immune system will attack it immediately. This much has been reported widely by the media. But important questions remain. How is mRNA actually synthesized as a transcription of the spike-producing segment of the virus' RNA? How is the selection and replication done? How does mRNA enter a host cell, and how long will it stay there? Will it produce the spike protein forever? Is it perhaps dangerous? And the biggest question of all: How does the immune system record the structure of the foreign protein, how does it recognize the invader, and how is the immune response cranked up? To answer these questions, we bring you a conversation between Ubiquity editor Walter Tichy and his daughter Dr. Evelyn Tichy, an infectious disease expert.
Computers were essential for sequencing the human genome in 2001. Since then, a symbiotic relationship between computing and biology developed. Once the genome data were in the computer, they were used in biomedical science (for instance, for protein structure prediction), anthropology, forensics, and other branches of science. Genomic studies have yielded advances in diagnosis and treatment of diseases and a better understanding of evolution. Computers are a central tool for all of this. Several information processes occur naturally in the immune system: copying or reproduction, error correction, massively parallel search, unique identifiers, challenge and response, agents, pattern matching, generate and test, translation, and memory. See whether you can identify these natural information processes in the following interview.
—Peter J. Denning, Editor-in-Chief
Walter Tichy: Let's start at the beginning. What is a virus and why is it dangerous?
Evelyn Tichy: Viruses are basically microscopic parasites. Unlike bacteria, viruses are not able to reproduce on their own. Viruses that infect humans hijack the body's cellular machinery to reproduce.
There are millions of types of viruses. They are found in almost every ecosystem on Earth and infect everything from animals and plants to bacteria. Most viruses that you have heard of infect and cause disease in humans, like cold sores (herpes simplex virus), warts (papilloma virus), Ebola hemorrhagic fever (Ebolavirus), the flu (influenza virus), or the common cold (which is caused by more than 200 different types of virus). There is a whole world of viruses out there that don't infect humans.
One of the things that I find absolutely fascinating is that a lot of symptoms of infections are actually side effects of your immune system fighting the virus. For example, when you are infected, your immune system increases your body temperature because many viruses' and bacteria's replication is temperature-sensitive. A fever slows down the multiplication of some of those pesky invaders. Of course, this can go horribly wrong if your temperature climbs too high and starts to cause serious damage to your body.
Viruses can also be dangerous because they can cause disease, not just in humans, but also in animals and plants. They can cause contagious diseases and difficult-to-control crop failures.
WT: Before we go into vaccination, it is probably a good idea to understand the immune system first. What happens if a new virus enters the body, and there was no prior vaccination?
ET: There's a lot to unpack in this question.
An infection is pretty much a war between the invading virus and the immune system defending against the infection. A vaccine gives the immune system a head start in reacting to the viral infection. To understand how this works let's start with what happens when a "new" virus, by which we mean one which your body has never encountered or "seen" before, enters the body. In order to reproduce, the virus needs to gain access to your body's cellular machinery because it doesn't have the critical equipment it needs to replicate itself. To do this, the virus invades and hijacks your cells. That means it needs to somehow recognize that cell, get inside, and then take over the existing replication machinery to make copies of itself. Those new copies can then go on to infect other cells, other hosts (your friends and family, the poor guy you sneezed on while riding the bus), and so on.
First, the virus docks on to your cells and then crosses the cell membrane. Virologists call these processes "attachment" and "viral entry" and they can be surprisingly complicated. Some viruses infect only very specific types of cells in your body, because they can only attach to very specific proteins on those cell surfaces. Attachment affects the symptoms of the disease that the virus causes. For example, HIV, the virus that causes AIDS, only infects a very specific type of immune cell, which ultimately leads to immune suppression and leaves AIDS patients open to lethal infections that their immune system would normally have no trouble fighting off. How good the virus is at attaching to and entering your cells is a big factor in how effective it is at infecting you.
How a virus attaches is also important because it helps determine which species it is able to infect. "Bird flu" is a type of influenza virus that originally infected birds, but not humans. It mutated to attach to both bird and human cells and now is able to infect both.
Once the virus is inside the cell it hijacks the existing cellular machinery to make copies of itself. This means that it makes lots of copies of its genetic code, uses your cells' ribosomes (the factories of your cells) to produce the protein building blocks of the virus' body, assembles new virus particles, and exits the cell in large numbers to infect more cells.
Now that we've understood what the virus does when it enters your body, let's have a look at what your body's defense system (the immune system) does to combat viruses and why your immune system is so much faster to react if it's seen a particular "bad guy" before.
The immune system is amazingly complex so I won't go into all the fascinating details, but I will give you an idea of what we don't yet know. Spoiler alert: There's a lot that scientists are still researching. Every time I turn around they seem to have discovered another fascinatingly clever component of defense in the body's fight against invaders.
Generally speaking, the immune system has three main lines of defense.
The first line of defense is the physical and chemical barriers that prevent entry or kill invaders. These include your skin and the mucosa of the respiratory and digestive tracts, which keep the bad guys out in the first place. The chemical barriers include some very clever compounds such as the antimicrobial lysozyme in your tears (yes, your tears can kill bacteria), and low pH conditions in your stomach that kill most things that do get in. Of course, pathogens have evolved all sorts of smart ways to get around those defenses. If you're interested in more in-depth reading, look up how the bacterium Helicobacter pylori, which causes stomach ulcers, neutralizes stomach acid.
Once a pathogen has successfully crossed the physical barrier and entered your body it encounters the second line of defense: the non-specific innate immune response. I like to think of the second line of defense as the "guards" or "spies" of the immune system. The second line of defense has two jobs: Kill anything that looks like it's not you (i.e. is probably an invader) and activate the third line of defense, which can then target the specific invader. There are lots of different types of cells that play a role in the second line of defense to protect you against all the different types of invaders that you might encounter—from macrophages to eosinophiles, mast cells, endothelial cells…—the list is long. A lot of these have evolved to detect specific types of bugs, for example, eosinophiles recognize and kill parasitic worms called helminths as well as protozoans, but aren't particularly useful against viruses.
Generally, these cells are found in your blood and tissues and try to kill anything that looks foreign. Phagocytes bind and engulf pathogens, break them up into pieces and then present the dead invader to the third line of defense for specific targeting and destruction. The other very important thing that the cells of the innate immune system do is recruit more immune cells to the site of the infection to help fight the invaders, which leads to an inflammatory response (swelling, redness, pain). There is a lot of really interesting research on how these cells migrate to the site of the infection, following the concentration gradients of various chemicals released by the cells in your tissues and lining your blood vessels.
The third and final line of defense is called specific adaptive immunity. This is probably the part of the immune system that you've heard the most about. It mainly involves two types of white blood cells (a.k.a. lymphocytes): B-cells which produce antibodies and T-cells that kill infected cells, damaged cells, and cancerous cells (like cellular assassins). The adaptive immune system is specific to individual molecules on individual pathogens (hence "specific" immunity) and, while very powerful, is slower to react than innate immunity as it must first be activated.
WT: B-cells, T-cells, antibodies, antigens—I'm a little confused. What do these cells do?
ET: Let's talk about B-cells and antibodies first.
Antibodies are Y-shaped proteins which are produced by B-cells and recognize or chemically bind to specific molecules on the surface of pathogens (these specific molecules that are recognized by the immune system are often called antigens). So, a specific antibody binds to one specific antigen. When an antibody has recognized an antigen, it can either neutralize the pathogen directly (e.g. by blocking parts of a virus that are essential for cell entry) or tag the pathogen or infected cell for attack by the other parts of the immune system, which we have already discussed. Once a B-cell is activated it uses a process called clonal expansion to generate large numbers of these specific B-cells against this specific antigen—a bit like building up an army that is very efficient at fighting a very specific enemy.
Human antibodies are classified into five types: IgG, IgM, IgA, IgE, and IgD (Ig = immunoglobulin = antibody). While each of these is designed to respond to different situations, the most common type in your bloodstream is IgG, which makes up about 70–75 percent of human antibodies.
T-cells' job is to destroy virus-infected cells, i.e., those cells of your body that have been hijacked by the viruses as well as cancerous or damaged cells. This function is performed by so-called "killer T-cells," which are activated when presented with a fragment of the pathogen, e.g. by a B-cell or dendritic cell "guard" from the second line of defense. T-cells then track down the cells infected by the virus and release cytotoxins, which lead to programmed cell death of the infected cell. Like B-cells, T-cells proliferate once activated to generate a large "army" capable of attacking only this specific enemy. There are all sorts of other types of T-cells, such as T-helper cells that regulate and enhance this function, but, fundamentally, T-cells induce infected, cancerous, or damaged cells to commit suicide to prevent further damage or infection.
The adaptive immune system, made up of B-cells and T-cells, is comparatively slow to react the first time a new pathogen invades the body, mainly because it needs to be activated and then build up its army of cells. However, the fantastic thing is that it can react really quickly and decisively if it has seen a specific invader before. This is called the "memory" of the immune system and it's a function that we exploit to create immunity using vaccines. So, if the adaptive immune system has "seen" the invader before (either as a vaccine or because you were infected by and beat the same bug as a kid) it can react quickly and with overwhelming force, preventing the infection from gaining a foothold in the first place. I like to think of the adaptive immune system as the "big guns": It takes time to get them ready but they're incredibly effective, especially if they're already entrenched, primed for the right bug, and waiting.
Now, this analogy of the three lines of defense of the immune system "army" is a very simplistic one when compared to reality. In fact, the immune system is more of a web of interlinked cascades, which is constantly modulating the strength and specificity of its response. After all, it doesn't make sense to deploy the tank brigade when you're being attacked by fighter jets and your immune system needs a way to back down once the invaders are dead.
WT: You mentioned recognizing and binding to invaders several times. How does that work? Your body has not seen an invader before, so what points out the bad guys?
ET: Recognizing "self" and "non-self" is one of the really interesting questions in immunology, particularly because when this goes wrong, i.e. when the body incorrectly recognizes "self" as "non-self" or foreign, it can result in what we call an autoimmune disorder, which is when the immune system attacks the body.
Self-identification is based on a group of identification molecules called human leukocyte antigen (HLA) or major histocompatibility complex (MHC). These function as an identification tag. They are located on the surface of all of the cells in your body in a combination that is almost completely unique to you. Any cell with molecules on its surface that are not identical to your HLA is recognized as foreign and destroyed by the immune system. These cells could be from an insufficiently matched transplanted organ or your own cells that are pushing out viruses. B-cells, which produce antibodies, can recognize invaders directly, but T-cells need help from antigen-presenting cells. (Remember our "guard" cells from earlier? This role can also be played by B-cells and dendritic cells). Antigen-presenting cells combine their own HLA molecules with fragments from the invader. This combination of HLA molecules and foreign antigen fragments is presented on the cell surface. A T-cell with a matching receptor on its surface can bind the HLA molecules presenting the foreign fragment, like a key fitting into a lock. This activates the T-cell, which can then fight the invaders.
WT: How does the T-cell come up with the matching receptor, which can "see" the HLA molecule and the "foreign" fragment, and how do B-cells come up with receptors to recognise antigens directly?
ET: Both B-cell and T-cell receptor diversity is randomly generated. This means that B- and T-cells with receptors capable of binding to unseen, future antigens are already present in your body. The number of possible receptors is large: about 3 x 1011 combinations are possible. This diversity allows for the recognition of antigens from nearly all pathogens including bacteria, viruses, parasites, worms, and some cancer cells. It is the defining feature of the adaptive immune system.
Due to random generation, antigen receptors that bind to your own cells are also produced. The adaptive immune system has to eliminate these cells to prevent your immune system from attacking your own body. This happens in the thymus, where the T-cells which respond to self are killed off in a process called negative selection. T-cells also undergo positive selection to ensure that they are able to recognise self. Any cells that do not recognise self are also killed off.
If you'd like to know more about the generation process, read the Wikipedia article on "V(D)J Recombination" or this article.
WT: This sounds a lot like generate-and-test in computer science: Randomly generating possible candidates and selecting those that work. What happens after a virus is fought off, and the immune system goes into a waiting state? How does it remember?
ET: Some of the B-cells turn into memory cells. Memory cells circulate in the body waiting for invaders that they have seen before to reappear. When this happens (e.g. if you are exposed to chickenpox for the second time or if you are exposed to a virus that you have been vaccinated against), they spring into action, quickly undergoing clonal expansion to generate an army of specific immune cells to fight off the invader before it gains a foothold. As a result, you don't get the symptoms of chickenpox a second time because your immune system fought it off before you even realized that you might be sick. There is a lot of ongoing research on the memory function of the immune system. For example, while we know that the memory immune response is much stronger than the initial, primary response, scientists still aren't sure why some vaccines result in stronger memory than others.
WT: How does a "traditional" vaccine, such as the flu shot, work?
ET: The fundamental idea behind a vaccine is to generate immunity to a specific bug. Vaccines do this by presenting the immune system with a harmless version of that invader, which activates the second and third line of defense of the immune system and generates memory cells.
Then, when you are infected with the actual, harmful version of that bug, your immune system can react more quickly and with overwhelming force, eliminating the threat before it can replicate in large numbers and cause the symptoms of the associated disease. Basically, vaccines give your immune system a head start so that it can clear the infection before it really gets started. If we revisit our army analogy from earlier, a vaccine makes sure that your immune system has seen the enemy before, knows how to defeat it, has built the right weapons in advance, and can react quickly.
WT: How long do immune cells survive? The cells of the body are replaced about every seven years.
ET: Well, it really depends on the type of immune cell, but, generally, weeks to decades (in the case of memory cells). Exactly how and how long memory cells survive is not yet fully understood.
WT: There are lots of different types of COVID-19 vaccines and some of them seem to be better than others. Why?
ET: There are lots of different types of vaccine. The world's first vaccine was the English physician Edward Jenner's smallpox vaccine. Smallpox is an extremely dangerous and highly infectious disease, an outbreak of which would kill about 10 percent of the population (and up to 20 percent in cities). Jenner postulated that milkmaids, who were generally immune to smallpox, were protected due to their infection with cowpox (a disease similar to smallpox, but much less virulent). Jenner tested this hypothesis by infecting his gardener's 8-year-old son, James Phipps, along with 23 other test subjects, with pus from cowpox blisters and then exposing them to infectious smallpox samples. This is called a live natural vaccine.
Vaccines prevent many millions of illnesses and save numerous lives every year. Modern vaccines come in many shapes and sizes and generally require many years of development and rigorous clinical testing. Let's have a look at some of the vaccines you may have heard of.
(Here are some excellent illustrations about how vaccines work. Download them and consult them while you read the following text.)
- Live, inactivated vaccines. Inactivated vaccines use the killed version of the bacterium or virus. They don't generate immunity that is as strong as live vaccines, so several doses (booster shots) may be required over time to maintain immunity.
Example vaccines: hepatitis A, rabies, COVID-19 (Sinopharm), COVID-19 (SinoVac), COVID-19 (Covaxin) - Live, attenuated vaccines. Attenuated vaccines are created by reducing the virulence of the bacterium or virus, by which we mean the ability to cause damage to the host (i.e. you and me). Generally speaking, attenuated vaccines produce a stronger and more durable immune response than inactivated vaccines, with a quick immunity onset.
Example vaccines: measles, mumps, and rubella (MMR), tuberculosis, yellow fever, chickenpox, smallpox (modern vaccine only) - Subunit, recombinant, polysaccharide, and conjugate vaccines. These vaccines use small pieces of the bacterium or virus, like proteins, sugars, or parts of the viral capsid, to activate the immune response. As they use specific parts of the pathogen, they elicit a strong immune response that is targeted to key parts of the bug. However, they often require booster shots.
Example vaccines: hepatitis B, human papillomavirus (HPV), whooping cough, meningitis - Toxoid vaccines. Toxoid vaccines create immunity against the toxin produced by the bug. The immune system is targeted against the toxin itself, not against the bug which produces the toxin.
Example vaccines: tetanus, diphtheria - mRNA vaccines. mRNA vaccines are a new type of vaccine, but researchers have been studying and working with mRNA vaccines for decades. mRNA vaccines are made up of messenger ribonucleic acid (mRNA), which is the genetic material for the component which triggers the immune system (the antigen). The mRNA instructs the cells of your body (such as the muscle cells at the site of injection) to produce large amounts of the antigen, which then triggers an immune response. The immune response destroys the antigen-producing cell and the mRNA contained in the original vaccine (mRNA is pretty unstable and is designed to degrade). The great thing about mRNA vaccines is that there is no risk of causing disease in the person getting vaccinated, because one is only injecting a small part of the virus.
Example vaccines: COVID-19 (Pfizer/BioNTech) and COVID-19 (Moderna) - Viral vector vaccines. Viral vector vaccines use a different, harmless, virus to deliver the genetic code for the component that triggers the immune system (the antigen), instead of the bug or antigen itself. By infecting cells and instructing them to make large amounts of antigen, which then triggers an immune response, the vaccine mimics what happens during natural infection with certain pathogens—especially viruses. This vaccine has the advantage of triggering a strong immune response by T cells as well the production of antibodies by B cells.
Example vaccines: COVID-19 (Oxford/Astrazeneca), COVID-19 (Johnson & Johnson), COVID-19 (Sputnik V), Zaire-Ebolavirus rVSV-ZEBOV
WT: Is an mRNA vaccine more dangerous than a traditional vaccine? Can I be infected? How long does it stay in the body? Is it replicated?
ET: An mRNA vaccine is much less dangerous than a traditional, live vaccine, because no actual virus is injected, so you can't be infected.
mRNA is generally quite unstable. In fact, one of the tricky things in the development of these vaccines was the instability of mRNA. If you're not careful in the lab, mRNA deteriorates in a matter of minutes to hours. As a vaccine, it must be protected by enclosing it in a tiny shell and it must be refrigerated to prevent degradation. The shell also helps with entering cells. In the body, mRNA is degraded by normal cellular processes. Its in vivo half-life can be regulated through the use of various modifications and delivery methods.
mRNA is generally not replicated. It serves as a set of instructions to produce proteins; in this case the SARS-CoV-2 spike protein. The cells containing the viral mRNA are eventually killed off by the immune system, because they produce the spike protein, which is a foreign protein. As a result, the viral mRNA is probably completely gone from your organism in a matter of days.
WT: How is an mRNA vaccine manufactured?
ET: One of the great things about mRNA vaccines is their potential for rapid, inexpensive, and scalable manufacturing. This is because the mRNA itself can be produced in vitro without needing human cell lines.
Roughly, manufacturing works as follows. First, the gene that produces the spike protein must be identified. One way to do this is to infect cells with pieces of the virus' genetic material until a cell that produces the spike protein shows up. If we know the genetic code of the virus, we can identify the individual genes by searching for "STOP" and "START" sequences and (partially) predict the structure of the encoded proteins from the genetic sequence.1 Next, the mRNA must be modified to make it more stable. This step involves designing protective caps for the ends of the mRNA sequence. (These days, this design work is all done on the computer, but I for one still learned how to do this using paper and pencil.) Next, one synthesizes the new, adapted mRNA in the lab. Techniques for producing large numbers of copies of the mRNA have been used by molecular biologists all over the world for many years. For small amounts, one can order the required chemicals as next day delivery. Finally, the synthesized mRNA molecules are enclosed in lipid nanoparticles or other protective shells.
WT: When you get an immunization shot, you may experience fever, swelling, and some pain at the point where the needle entered. Is that a bad sign?
ET: No, this is actually a sign that your immune system is doing its job! The swelling and pain are because all of those second line of defense immune cells are rushing to the injection site. Increased blood flow makes it easier for the immune system to get its army into the field. As we talked about earlier, the body increases its core temperature in an attempt to kill off the invaders (some of them can't survive or can't reproduce at higher temperatures).
That being said, a very small number of people do have adverse reactions to some vaccines or their components. The risk of having a serious adverse reaction to a modern vaccine is incredibly low and, in the case of the coronavirus, much much lower than the risk of suffering lasting damage or death as a result of catching that virus.
WT: Lots of people are afraid that immunization causes autism. Is that true?
ET: No. This is completely fake news! The myth that vaccines cause autism is based on a fraudulent and completely discredited research paper by British gastroenterologist Andrew Wakefield. It links the measles, mumps, and rubella (MMR) vaccine to developmental disorders in children and was published in 1998. The fraud was exposed in 2004 by journalist Brian Deere and is considered by some to be the most damaging medical hoax of the 20th century. Wakefield lost his medical license and his article was retracted by Lancet.
Several other major studies were conducted to investigate Mr. Wakefield's claims and none of them found any link between vaccines and autism.
I, for one, had my COVID-19 vaccination as soon as it was my turn.
WT: Every few months a new coronavirus variant appears and they seem to be getting more dangerous. Why does the virus mutate so rapidly, and what does that mean for vaccinations?
ET: Actually, this is not the case. In the grand scheme of things, this coronavirus doesn't mutate as fast as it could, gaining mutations at about half the rate of the influenza virus and about a quarter the rate of HIV. This is probably due to the presence of a so-called proof-reading enzyme, which checks for errors (aka mutations) during viral RNA replication. The issue, of course, is that millions of people have been infected, so, while we're very lucky that this virus mutates comparatively slowly, the sheer number of infections means we will continue to see the emergence of dangerous new mutations.
Mutations can be dangerous for a number of reasons: either because the direct effect of the mutation makes the virus more infectious (for example, mutations that make it easier for the virus to enter human cells), or produces more detrimental symptoms, or because the mutation decreases the efficacy of our vaccines. This can happen when the part of the virus that is recognized by the immune system (the antigen) mutates and looks different, so that the probability of a match is lower. Coming back to our lock-and-key analogy: Our perfectly fitting key has changed its shape and fits poorly or not at all. Vaccine efficacy decreases depending on how poor this match is, which is why we often talk about percentage effectiveness of vaccines against virus variants.
As the pandemic progresses, dangerous mutations will continue to emerge and will outcompete the original, less harmful variants, which is why we need to vaccinate as quickly as possible and get the case numbers down, simply to decrease the probability of this happening.
WT: There is speculation that the virus causing COVID-19 jumped from animals to humans at a wet market in Wuhan. How does this work?
ET: We don't actually know where exactly the coronavirus came from. However, we do understand how viruses from animal hosts can jump to human hosts (for example, bird flu, which is caused by the avian influenza virus).
Generally speaking, human influenza viruses infect humans and originally, avian influenza viruses infected birds. However, both can infect pigs. If a pig is infected by both viruses simultaneously, something called "antigenic shift" can occur: The viruses can mix their genetic material during replication. This is called "reassortment" and can result in a new influenza virus subtype, which has most of its genes from the human influenza virus and some from the avian virus. Reassortment can also happen if the avian virus mutates to infect humans, which today's bird flu managed.
If we're unlucky, this new virus is more infectious and causes more severe symptoms than the original human influenza virus, while also being "unknown" to our immune system's memory—a dangerous combination.
WT: Thank you, Evelyn, for this highly informative interview!
Author
Evelyn Tichy is an international public health and infectious disease expert working for one of Germany's largest COVID-testing providers. Previously, she spent a stint at the WHO assessing the response to the Ebola crisis. She holds a Ph.D. in biochemistry from the University of Cambridge, UK, and a Diploma in microbiology, virology, and immunology from the University of Tübingen, Germany. In her spare time, Evelyn enjoys messing about in boats.
Walter Tichy has been professor of computer science at Karlsruhe Institute of Technology in Karlsruhe, Germany, since 1986. His research interests include software engineering, parallel computing, and artificial intelligence. He is best known for his work in software configuration management and empirical studies of programmers. Before Karlsruhe, he was assistant professor at Purdue University. He holds a Ph.D. in computer science from Carnegie-Mellon University. In his spare time, he plays the grand piano.
Footnotes
1As a side note, there are some really exciting recent developments on the intersection between biochemistry and computing here. The ability to accurately predict a protein's structure from its sequence reduces the time needed for structure determination from potentially several years to hours. This means that, as biochemists, we can concentrate on studying the function of the protein as opposed to spending our time growing crystals for analysis at the synchrotron.
©2021 ACM $15.00
Permission to make digital or hard copies of all or part of this work for personal or classroom use is granted without fee provided that copies are not made or distributed for profit or commercial advantage and that copies bear this notice and the full citation on the first page. To copy otherwise, to republish, to post on servers or to redistribute to lists, requires prior specific permission and/or a fee.
The Digital Library is published by the Association for Computing Machinery. Copyright © 2021 ACM, Inc.
COMMENTS